Jie Shan and Kin Fai Mak: Uncovering Quantum Worlds With 2D S’mores
Jie Shan and Kin Fai Mak, a married couple of condensed matter physicists at Cornell University, uncovered a variety of new quantum phenomena by stacking layers of 2D crystals into s’mores.
Lindsay France / Cornell University
Jie Shan, left, professor of applied and engineering physics in the Cornell College of Engineering, and Kin Fai Mak, assistant professor of physics in the Cornell College of Arts and Sciences, are a married couple of physicists who enjoy collaborating in the lab.
This article is adapted from my article in Bronx Science’s 2023 physical science magazine, REACTIONS.
Sitting around a campfire, laughing with friends, and roasting marshmallows on sticks is the quintessential way to enjoy the summer. But don’t eat the marshmallow by itself: make a s’more. The trick is to take a marshmallow, a hunk of chocolate, and sandwich them between layers of graham crackers. By stacking the pieces into a s’more, you can taste the distinct flavors of each ingredient dissolve in your mouth, creating a new flavor entirely. Luckily, the same principle behind delicious s’mores is starting to fuel quantum leaps in physics.
Jie Shan and Kin Fai Mak, a married couple of condensed matter physicists who run a joint research group at Cornell University, have successfully stacked layers of 2D (or atom-thick) crystals that display a variety of novel quantum phenomena. A mishmash of forces and properties come together in these s’more melting pots. The group has coerced the particles to merge into a quantum fluid and freeze into a collection of icelike structures. They’ve assembled huge artificial atoms that are acting as test beds for fundamental theories of quantum matter. Theorists say the couple’s work broadens our understanding of what flocks of electrons are capable of. “It’s a crazy playground,” said James Hone in Quanta Magazine; Hone is a researcher at Columbia who supplies the Cornell lab with high-quality crystals. “You can do all of modern condensed matter physics in one material system.”
Graham Cracker — Laying the 2D Plane
In general, you can expect a material’s properties on the macroscopic scale to reflect what its electrons are doing on the microscopic scale. In conductors like metals, electrons buzz from atom to atom, carrying watts of electricity. In insulators such as wood and rubber, the material thwarts any electrons from infiltrating. Meanwhile, semiconductors such as copper and carbon are somewhere in the middle: their electrons can flow with the influx of energy, making them ideal for switching currents on and off — the role of a transistor. For the longest time, these were the essential electron behaviors though to describe the properties of most materials.
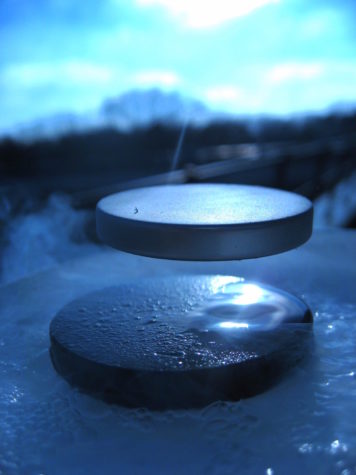
But in the last century, physicists have observed electrons behaving in more exotic ways. When most metals are cooled to frigid temperatures within a few degrees of absolute zero, their electrons pair up and form a type of quantum fluid. Once this happens, electrons no longer interact with atoms in the material — interactions that generate resistance — which allows them to flow with no energy loss whatsoever. This dramatic transformation of electrons, known as superconductivity, occurs around the ‘critical temperature’ of the material. In the 1980s, the phenomenon was observed in copper oxide crystals (cuprates) at 35 Kelvins. Later, scientists would upgrade that number with the discovery of more cuprates that superconduct at temperatures as high as 100 Kelvins.
The upwards trend in critical temperatures inspired a dream in scientists for the overarching goal of condensed matter physics: the engineering of a substance that can superconduct in our room-temperature, nearly 300-Kelvin world. The hypothetical benefits to superconductive technology are vast and unending: enabling lossless power grids, levitating vehicles, and hyper-efficient devices that could significantly reduce humanity’s energy needs. But the extreme conditions needed to unravel superconductivity have so far prevented it from stirring up a technological utopia.
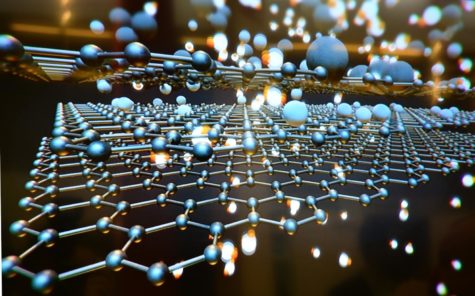
This was the reality of superconductivity until in 2004 when two researchers at the University of Manchester, Andre Geim and Kostya Novoselov, made a groundbreaking discovery using two familiar household items: graphite and Scotch tape. They found that the tape could be used to peel off strips of graphite into sheets one atom thick. It turned out that these crystalline sheets, a honeycomb lattice of carbon atoms a million times thinner than a human hair, held astounding properties absent from their three-dimensional crystal counterparts.
Graphene, as it came to be called, quickly became the world’s ‘wonder material.’ It’s the strongest fabric out there — stronger than diamonds, 200 times stronger than steel. Graphene was also the world’s first flat material — a plane on which electrons can whiz by at a million meters per second, but not up or down. What’s intriguing is that the electrons didn’t seem to slow down once they got caught up in the lattice. Electrons flew as if they had no mass at all, granting the material a blistering degree of conductivity. Sometimes, this conductivity made graphene a difficult topic of research in the lab. Still, graphene’s discovery dubbed a new category of substances — 2D materials. The promise of future innovations from 2D materials won its discoverers the Nobel Prize for Physics in 2010.
Marshmallow — Roasting Energetic Excitons
That same year, as flocks of condensed matter physicists began studying graphene, Shan and Mak, then two young physicists at Columbia University, saw signs that the mineral molybdenite, a derivative of molybdenum sulfide, might be even more profound than graphene. The crystal looks almost identical to graphite to the naked eye, but on the particle level, its atomic makeup could not be more disparate. However, molybdenite held properties too difficult to study in a typical lab — still, it captivated Shan and Mak. The persistent duo has devoted nearly a decade of research fiddling with 2D molybdenite and a family of closely-related 2D crystals known as transition metal dichalcogenides, or TMDs.
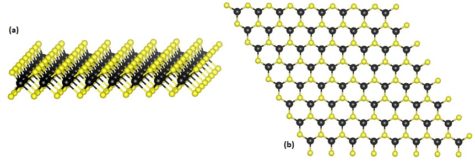
Now their effort shows enormous success. Shan and Mak’s research group at Cornell has discovered a plethora of strange electron phenomena in flat crystals of molybdenum disulfide and its TMD relatives. They did this by stacking sheets of different TMD lattices on top of another, and since the layers sit a fraction of a nanometer apart, the electrons interact like it were one substance. And unlike graphene which is exclusively strung together by carbon atoms, each TMD lattice is composed of a unique assortment of elements, bringing new flavors and interactions to the stacking game. The result of this novel mixing-and-matching of materials is that you can observe new emergent properties over the whole s’more that you wouldn’t observe in a single layer. Some s’mores are magnetic, others are superconductive.
Moreover, the stacking of 2D materials represents the realization of a long-held aspiration among condensed matter physicists. No longer are researchers limited to materials found on the ground or grown slowly in the lab. Now they can play with the atomic equivalent of s’mores, tweaking the layers to build custom structures with the desired flavors. Shan and Mak fashion their materials to observe the TMD’s combined powers.
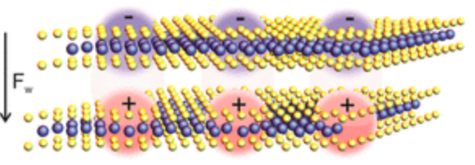
The duo’s first major discovery at Cornell had to do with excitons. You see, electrons aren’t the only thing speeding through the lattice — holes, the small inlets of emptiness created when electrons hop to-and-fro, spot the material as well. When extra electrons in one layer become attracted to positive holes in the layer below, the result is a beam of energy in the fleeting moment before the electron seals the hole. Shan and Mak measured the force of attraction between electron-hole pairs in 2D tungsten diselenide and found that it clocked in at hundreds of times stronger than the same pairs in a typical 3D semiconductor. The finding suggested that excitons in TMDs were especially potent, and that in general electrons are likely to act in odd ways.
Excitons are exciting for physicists because they offer a new avenue of quantum research that could culminate in room-temperature superconductivity. As quasiparticles, excitons exhibit the same features as electron-electron pairs, meaning that these electron-hole pairs become bosons, which allows them to condense into a quantum state known as the Bose-Einstein condensate. At temperatures scraping absolute zero, this rare state of matter could display quantum properties such as superfluidity, the ability of a fluid to flow while dodging any resistance (when a superfluid carries an electrical current, it superconducts).
Still, there are problems with this model. Unlike repellant electrons, electrons and protons are swift to join up. Challenges to exciton-based superconductivity lie in keeping the electrons from plugging the hole, and getting the electron-hole pairs to flow along a current — ideally, in as warm a room as possible. So far, in 2019, Shan, Mak, and their group at Cornell have been able to squeeze out exciton condensation at 100 Kelvins. Using s’mores of TMDs, excitons held on for tens of nanoseconds, a typical lifetime for this quasiparticle. Then, in 2021, the group built an improved apparatus that upped that number to a few milliseconds — a billion-fold improvement, which Mak remarked was “practically forever.”
Chocolate — Moiré Materials Strike Gold
Another graphene breakthrough inspired a second physics revolution. In 2018, Pablo Jarillo-Herrero, a researcher at MIT, showed that twisting layers of graphene relative to the layer below created new kinds of 2D materials. The idea was that you could drop an upper layer of 2D hexagons at a slight twist, a 1.1° rotation with the hexagons below. The angle misalignment results in a structural offset which oscillates at regular intervals throughout the material, creating large patterns of ‘supercells’ known as a moiré superlattice. At the ‘magic angle’ of 1.1°, the unique crystal structure of the s’more would force graphene’s electrons to halt and sense the repulsion of nearby electrons.
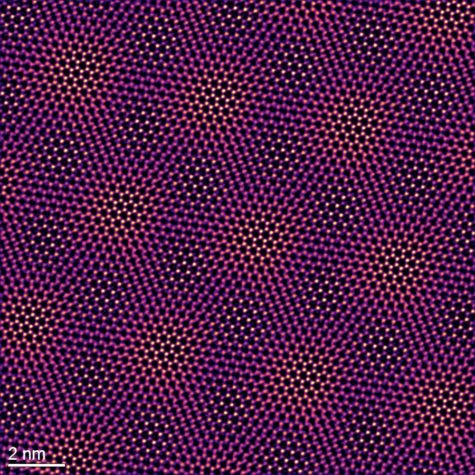
Electrons tend to go out of character when they become aware of one another in this way. You see, in normal insulators, electrons undergo quantum tunneling, a maneuver comparable to teleportation, which lets them move too quickly to interact with each other. However, in a moiré lattice, this phenomenon rarely occurs because supercells sit roughly 100 times further apart than the atoms that comprise them. Slowed to a halt, the electrons then get to know their neighbors. Jarillo-Herrero had shown in a series of experiments that, for unknown reasons, this electron-to-electron communication can give rise to especially potent superconductivity.
What’s more, at the magic angle, electrons seemed to go blind to the individual lattice atoms and experienced the large networks of supercells as if they were on the scale of atoms. With enough electrons, the supercells could exhibit collective quantum states. Using an electric field to adjust the number of electrons in each supercell, Jarillo-Herrero’s group was able to create a superconductor, an insulator, and a number of other elusive electron behaviors from their twisted bilayer graphene device.
Although graphene’s twisted moiré superlattices provide a radical new way to control electrons, experiments with 1.1° misalignments hinged on an arbitrary argument — what reason do atoms have to fall neatly into the 1.1° misalignment? The budding field of ‘twistronics’ lacked a conclusive answer.
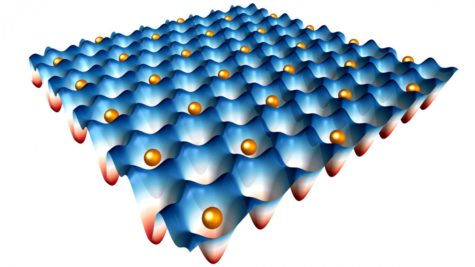
In search of better answers, Shan and Mak use TMDs as an alternative for creating moiré superlattices. Because each TMD has a hexagonal lattice of a different size, stacking lattices of larger hexagons over smaller ones produces a moiré pattern equivalent to that of an angle misalignment, without the need for chance. TMDs are also ideal for exploring moiré electron interactions. They are semiconductor materials, so their electrons are constrained as they move through the lattice, unlike the uncontrollable conductivity found in graphene. The research group at Cornell would soon discover electrons crystalling into almost two dozen repeating patterns known as Wigner crystals on TMD superlattices — an indication of the potential of TMD moiré s’mores.
At the same time, Shan and Mak’s team of researchers were creating TMD moiré materials that could simulate an important framework of condensed matter physics — the Hubbard model. Proposed by Martin Gutzwiller, Junjiro Kanamori and John Hubbard in 1963, the model is physics’ best attempt to capture the endless variety of crystalline superlattices to their most fundamental aspects. It supposes that each electron is torn between two competing forces: it wants to move to neighboring atoms by tunneling, but it’s also repelled by its neighbors at the same time. Different behaviors emerge when this equilibrium of forces is interrupted. The only problem is that the Hubbard model has been mathematically unfeasible to solve except for the case of a 1D string of atoms.
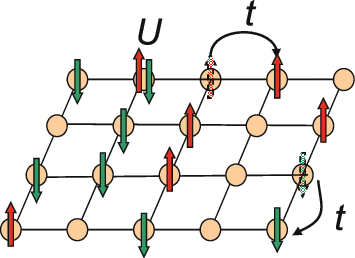
Instead of scratching their heads at impossible equations, Shan and Mak use moiré superlattices to gauge what nature has to say about a 2D Hubbard model. To build their simulation, they stacked layers of tungsten diselenide and tungsten disulfide, and they attached electrodes that could tune up or down the electric field passing through the system. With more electrodes, the team could extend the force of attraction for each supercell on the lattice, analogous to adding positive protons to the nucleus, thereby making it harder for negative electrons to tunnel away. This way, the electric field controlled how many electrons would populate each supercell.
And since each supercell acts like its own giant atom, going from one electron to two electrons per supercell is like transforming a lattice of hydrogen atoms into a lattice of helium atoms. So far, the simulation is able to reproduce elements with up to eight atoms, from hydrogen to oxygen. In some sense, these designer atoms recall the ancient aim of alchemists to conjure gold out of thin air. One by one, each element of the periodic table could have a moiré material counterpart. In principle, this Hubbard model simulator can even invoke fictitious atoms found nowhere in nature with, for example, 1.57 electrons each.
Shan and Mak’s simulation of the Hubbard model was complete. The TMD moiré system lets them customize the atomic playing field, even into systems that don’t exist in nature, and smoothly transform them with the sway of parameters. It’s a power that borders on profound, illuminating the possibility of quantum physics research transpiring on these grids. “If I were to single out their most exciting and impressive effort, that’s the one,” said Philip Kim to Quanta Magazine; Kim is a condensed matter physicist at Harvard University.
Although the current simulator doesn’t have free reign over the parameters, it has shown promise by settling a 70-year-old debate: What if you took an insulator and converted its atoms into a conducting metal, would the transition happen gradually or abruptly? Translating the components into the lab, Shan and Mak were able to carry out the thought experiment.
First they simulated heavy atoms, which trapped electrons so that the TMD s’more acted like an insulator. Then they shrank the atoms, weakening the deadlock until atoms were able to bounce about, letting the s’more become a conducting metal. By observing a gradually falling electrical resistance as the superlattice acted more and more like a metal, the researchers showed that the transition, known as the Wigner-Mott transition, was not abrupt. The finding opens up the possibility that the superlattice’s electrons may be able to display a long-hypothesized type of fluidity known as a quantum spin liquid. “That may be the most interesting problem one can tackle,” Mak said.
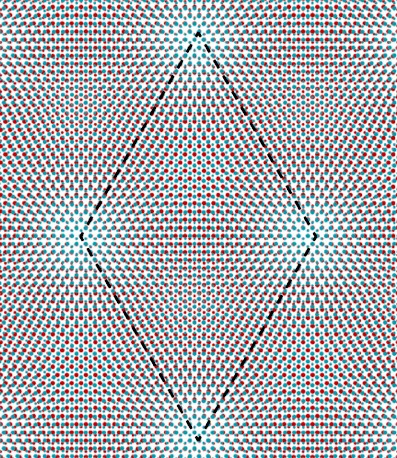
But this is not the end of the anomalies. As they were working on the Hubbard simulator, Shan and Mak’s team used TMD layers in which the hexagons on the two layers are aligned one-to-one, with transition metals atop transition metals and chalcogenides atop chalcogenides. Then, serendipitously, the team repeated the experiment in which the top layer had been stacked backward. The outcome of this accidental slip-up were bewildering — in some areas, resistance fell as electrons began tunneling, but in others, the resistance fell abnormally low, almost as if the material had superconducted. The researchers detected an obscure pattern of resistance known as the quantum anomalous Hall effect, evidence that something mysterious was going on here.
The effect indicated that the crystal structure of the device was compelling electrons along the edge of the material to act differently from those in the center. In the middle of the device, electrons were trapped in an insulating state. But along the edges, electrons walked the perimeter, almost like as if they were dancing around the centerpiece. By accident, the researchers had observed an extremely unusual type of matter known as a Chern insulator.
The quantum anomalous Hall effect, first observed in 2013, usually collapses if the temperature rises above just a few hundredths of a Kelvin. But Shan and Mak believe, with some tinkering, they can build Chern insulators at up to 50 or 100 Kelvins. If the researchers succeed, the discovery could lead to another way to get current flowing without resistance, at least for nanowires, which the Chern insulators might be able to transform how energy is streamlined through a device.
Graham Cracker — Technologies of the Future
Even as their achievements pile up, the couple shows no signs of stopping. Shan and Mak haven’t even begun experimenting with the weirder TMDs. After spending decades inventing the necessary equipment to explore the universe of 2D materials, they’re finally preparing to reach beyond their foundations in molybdenum disulfide back in 2010. Mak recently received a $1.25 million grant from the Moore Foundation Experimental Physics Investigators Initiative last fall to further their research.
The two researchers attribute their success to a culture of cooperation they experienced at Columbia. Today the duo foster a similarly relaxed environment at Cornell, where they oversee a couple dozen postdocs, visiting researchers and students, all of whom are largely free to go at their own pace. “Students are very smart and have good ideas,” Mak said. “Sometimes you don’t want to interfere.”
When asked about what excites them most regarding the future of their fields, Shan told Cornell Chronicle, “As we get to know more about what’s going on in other fields, and with other materials, our hope is that we’ll be able to maybe utilize, introduce these new elements to research collaborations with colleagues.”
Their marriage also makes their lab unique. Aside from her brilliance as an experimentalist, Shan has a careful discipline that makes her a good manager, whereas Mak is prone to spells of enthusiasm that push him deep into the technicalities. Mak enjoys toiling alongside the early-career researchers, both inside and outside the lab. He recently started rock climbing with the group. “It seems like their lab is their family,” said Andrea Young, a colleague of Mak’s during his grad years at Columbia University (now a professor at the University of California, Santa Barbara).
The devices that Shan and Mak are building may stack up to be more than a sum of their parts. As researchers wedge TMD sheets to create moiré superlattices and excitons, they speculate about how the new ways of controlling electrons might undergird future technologies. Even if the prospects of superconductivity fueling our power grids within our lifetimes remain nebulous, Bose-Einstein condensates could lead to ultra-sensitive quantum sensors, and better control of Chern insulators could someday enable powerful quantum computers.
And those are just the glowering cliffs on the technological horizons. Incremental improvements in materials science often add up to innovative applications few can anticipate. The researchers who developed the transistor in the late 1940s, for instance, would not have foreseen smartphones powered by millions of microscopic switches stuffed into a chip the size of a fingernail. Nor would the scientists who endeavored to fashion glass fibers that could carry light across their lab bench have been able to predict that 10,000-kilometer undersea optical fibers would someday tether continents. It’s much more likely that applications will generate on their own once the platform for development has been established. Two-dimensional materials may evolve with similarly explosive growth.
Shan and Mak’s landmark research might reflect the longstanding movement to adapt technologies to an increasingly quantum world. Headlines featuring terms such as quantum computing, quantum entanglement, or quantum simulations frequently float to the top of science newsletters. Talk among physicists over the potential to investigate quantum interactions reanimate with continued optimism and intrigue. Richard Feynman, a theoretical physicist who won the Nobel Prize in 1965 for his contribution to quantum electrodynamics, entertained the excitement surrounding quantum technology in a 1981 lecture, “Nature isn’t classical […] and if you want to make a simulation of nature, you’d better make it quantum mechanical.”
It will take radical shifts in technology to fulfill the scope of Feynman’s words. It will take grappling with tough problems, and it will take ingenuity to solve them. Most of all, it will take a synergy of quantum states to accomplish goals unreachable by only one or the other — like the flavors of a s’more on a hot summer night, or the partnership of two motivated researchers. “One plus one is more than two,” said Mak.
The devices that Shan and Mak are building may stack up to be more than a sum of their parts. As researchers wedge TMD sheets to create moiré superlattices and excitons, they speculate about how the new ways of controlling electrons might undergird future technologies. Even if the prospects of superconductivity fueling our power grids within our lifetimes remain nebulous, Bose-Einstein condensates could lead to ultra-sensitive quantum sensors, and better control of Chern insulators could someday enable powerful quantum computers.
Rajin Tahsan is a Copy Chief for ‘The Science Survey.’ Rajin enjoys journalistic writing because it enables him to explore fascinating intersections...